|
|
|
The Chemist Volume 91 | Number 2 |

|
|
Physical Chemistry of Environmental Interfaces:
Aerosols, Nanomaterials & Indoor Surfaces
|
|
Abstract: The 2018 Chemical Pioneer Award symposium provided an opportunity to give an overview of the physical chemistry of environmental interfaces. These interfaces include atmospheric aerosols, nanomaterials in the environment and indoor surfaces. As discussed below, detailed physical chemistry studies on these complex surfaces are challenging yet can provide important insights into processes occurring on interfaces in various indoor and outdoor environments.
Key Words: Environmental interface, Aerosol, Nanomaterial, Indoor surface.
|
|
|
Overview of Award Address
This article provides a brief summary of the Chemical Pioneer Award address entitled “Physical Chemistry of Environmental Interfaces: Aerosols, Nanomaterials and Indoor Surface”, presented on May 10, 2018 at the annual meeting of the American Institute of Chemists held in Philadelphia at the Science History Institute. The focus of this address was on environmental interfaces. Environmental interfaces are defined here as any surface in equilibrium with its surrounding environment. From this broad definition, there is a myriad of different types of environmental interfaces that include atmospheric aerosols, nanomaterials, and indoor surfaces.
The physical chemistry of environmental interfaces puts an emphasis on molecular and nanoscale level understanding of the detailed interactions that occur in these inherently complex systems. Examples of the complexity of these interfaces and how a deeper understanding can be obtained through a physical chemistry, molecular-based studies approach were highlighted in the award address.
Environmental Interfaces: Atmospheric Aerosol Surfaces
Atmospheric aerosols which are defined as solid or liquid particles suspended in air with diameters between about 0.002 μm (2 nm) to about 100 μm. Aerosols are an important component of the Earth’s atmosphere and come from a variety of different sources. This is a large size range (5 orders of magnitude) which reflects the large difference in sources and formation mechanisms. There is also a large range of very different chemical compositions across this size range.
Two main primary sources of atmospheric aerosols include desert regions and oceans, which give rise to mineral dust and sea spray aerosols, respectively. Once lofted into the air, the surface of these two aerosols can interact with atmospheric gases, including water vapor and trace gases. Focusing on sea spray aerosol, recent studies have shown the complexity of SSA which on an individual aerosol particle level contains a mixture of salts, organic compounds and biological components [1-3]. The relative amounts of each of these depend on particle size and formation mechanism, whether formed through jet or film drops [4]. Recent studies have focused on understanding the physicochemical properties of this important component of the Earth’s atmosphere.
Figure 1 depicts some recent developments from single particle analysis in determining composition from electron microscopy [2], Raman microspectroscopy [3] and reactivity studies [5]. For reactivity studies, the reaction of nitric acid with sea spray aerosol was investigated. The current paradigm for the formation of particulate nitrate in the marine boundary layer involved the well-known reaction of the nitric acid gas with sodium chloride in sea spray aerosol, as shown in reaction (1):
HNO3 (g) + NaCl (aq) → NaNO3 (aq) + HCl (g,a) (1)
However, little was known about how other components within sea spray aerosol reacted with this trace gas in the atmosphere. For these reactivity studies, both authentic and model systems for sea spray aerosol were exposed to the nitric acid vapor. Two new reaction pathways were found and involved, carboxylate and phosphate groups present within lipopolysaccharides (LPS) in the aerosol. These reactions can be written as follows:
HNO3(g) + R-COONa(a) → NaNO3 + R-COOH (2), and
HNO3(g) + R-HPO4Na(a) → NaNO3 + R-H2PO4 (3).
Kinetics for these reactions differ with the rate of reaction for LPS [reactions (2) and (3) being three to four times slower than that of NaCl (reaction (1)].
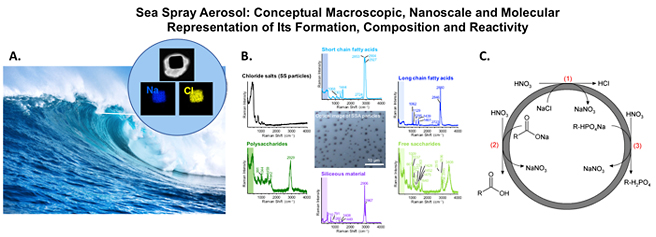
Fig 1. Sea spray aerosol, produced from wave action and bubble bursting at the ocean-atmosphere interface, is composed of salts, organic compounds and biological components. Understanding the physical chemistry of sea spray aerosol requires a single particle analysis approach using different microscopic and spectroscopic probes to unravel the nanoscale and molecular representation of the composition and reactivity. A. Electron micrograph identifying with energy dispersive electron spectroscopy the inorganic core of a sea spray aerosol particle with an organic outer coating; B. Raman spectroscopy identifying organic and biological components of sea spray aerosol and; C. Reactivity studies of sea spray aerosol identify new reaction pathways with nitric acid and sea spray aerosol due to the various functional groups within different components of sea spray aerosol. |
Environmental interfaces: Nanomaterial surfaces and their interaction with atmospheric gases
Nanomaterials have high surface to volume ratios. This means that the energetics and properties are controlled to a large extent by the surface. Given that the surface plays such an important role in the properties of nanomaterials, it is important to understand how common nanomaterials, e.g. metal oxide nanoparticles, interact with atmospheric gases.
In a recent tutorial review, Mudunkotuwa and Grassian provided insights into how nanomaterials transform in the environment [6]. In particular, several examples of nanomaterial transformations that can occur
in atmospheric and aqueous phase environments was laid out which show a wide range of transformation are indeed possible. In atmospheric environments metal nanoparticles can be easily oxidized to form metal oxide nanoparticles. In the case of CU nanoparticles, these nanoparticles change over time [7]. Initially, the nanoparticle surface region is oxidized on top of a metal core. This core shell morphology changes over time as the metal core shrinks and the oxidized layer grows with time due to oxidation with atmospheric oxidants including molecule oxygen. Additionally, it has been found the oxidized layer is composed of two phases,
CU2O and CUO, with the most oxidized layer CUO at the outer surface of the particle.
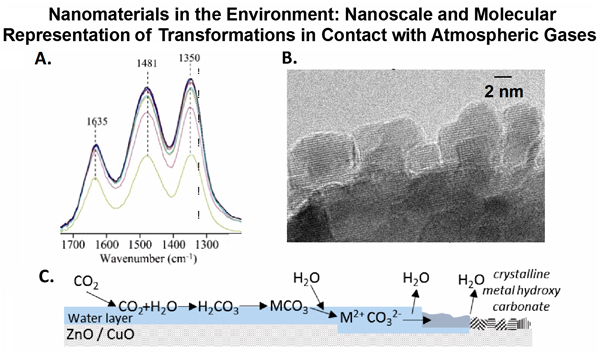
Fig 2. 20 nm CuO nanoparticles, as does ZnO nanoparticles, form metal hydroxy carbonates upon exposure to environmentally relevant concentrations of carbon dioxide and water vapor. A. The infrared spectra of CuO surfaces change while being exposed to CO2 and H2O. B. Analysis of these surfaces with transmission electron microscopy show the formation of crystallites which for CuO is identified as
Cu2(OH)2CO3Cu3(OH)2(CO3)2 . C. A pictorial schematic of this chemistry is shown for both CuO and ZnO. Most interesting, this chemistry with atmospheric gases causes the particles to be more soluble leading to higher concentrations of Zn2+ and Cu2+ when these nanoparticles are dispersed in water. Reprinted with permission from Gankanda et al. 2016 (Ref. 8). Copyright 2016 American Chemical Society. |
For metal oxide nanoparticles, the surface of these particles can undergo surface chemistry with trace atmospheric gases that change the properties of the nanoparticles. An example of this comes from Gankanda et al. [8], where reactions of 20 nm ZnO and CuO nanoparticle surfaces with carbon dioxide and water vapor. When adsorbed simultaneously, the nanoparticles were found to be more soluble in aqueous systems.
Figure 2A shows the results from spectroscopy of CuO nanoparticle surfaces in the presence of carbon dioxide and water vapor at environmentally relevant concentrations. These spectra were recorded as a function of time. It can be clearly seen there is adsorbed water (1635 cm-1 band due to the water bending mode) and carbonate ions (1481 and 1350 cm-1, due to the splitting of the asymmetric stretch), which are water solvated [9], on the surface of these nanoparticles. The surface reaction of CuO with these atmospheric gases is irreversible and transmission electron microscopy reveals the presence of small crystallites identified as copper hydroxy carbonates which form on the particle surface (Figure 2B). Similar reactions occur for ZnO nanoparticle surfaces when exposed to CO2 and H2O. The formation of these metal hydroxy carbonates occurs only when nanoparticles are exposed simultaneously to CO2 and H2O as shown in the mechanism depicted in Figure 2C.
Environmental interfaces: Indoor surfaces and their interactions with organic compounds
It is increasingly clear that surfaces impact indoor air chemistry and indoor air quality. New molecular-based research in this area is necessary in order to fully understand processes occurring on surfaces on a molecular and nanoscale level. Many of the challenges and outstanding questions regarding the chemistry of indoor surfaces have been enumerated in several excellent review articles (see e.g. Ref. 10). Moving forward, this area will require new approaches and new methods.We have recently begun a two prong approach to better understanding indoor surface chemistry which involves both fundamental studies of the adsorption and reactivity of indoor surfaces with organic compounds and oxidants and the placement of indoor surfaces in different local indoor environments and analyzing these surfaces with advanced microspectroscopic imaging techniques.
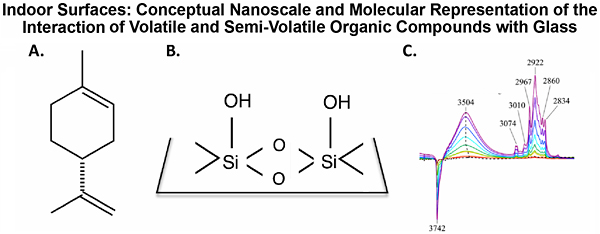
Fig 3. Infrared spectroscopy is being used to better understand the kinetics and thermodynamics of the adsorption of organic compounds on indoor surfaces. (A) Limonene and (B) SiO2, glass - a common surface found in indoor environments can interact as shown in (C) the infrared spectra as a function of increasing limonene pressure. |
Focusing on glass surfaces, a ubiquitous indoor surface, we are investigating the physical chemistry, including kinetics and thermodynamics of surface adsorption and desorption, of relevant organic compounds. These studies have begun with d-limonene, a common cyclic terpene found in indoor air. Sources of d-limonene include cleaning products and as an odorant in a variety of consumer products [11]. Figure 3 shows the molecular representation of limonene (A) and surface hydroxyl groups on glass surfaces (B) along with the FTIR spectra of limonene adsorption on silica as a function of increasing gas phase concentration. What can be clearly seen in the spectral region shown (OH and CH stretching regions) is that the absorption band intensities increase as more limonene adsorbs on the surface and that the OH groups are involved in the interaction. This can be observed as a decrease in the intensity of the isolated Si-OH groups seen at 3742 cm-1 as they hydrogen bond to adsorbed limonene resulting in a broad OH absorption band present at 3504 cm-1. Other absorption bands in the spectra are associated with adsorbed limonene (gas-phase absorptions are subtracted from the spectra).
The nature of this hydrogen-bonding interaction is unclear and the impact of relative humidity (i.e., water vapor) on the adsorption of limonene and other indoor air relevant organic compounds need to be better understood. Furthermore, there needs to be a better understanding of the adsorption/desorption kinetics as well as surface reactions with oxidants found in indoor environments. Studies that address these issues will provide additional insights into indoor air chemistry, these are all currently underway.
Future Outlook
Environmental interfaces are complex by their very nature. This complexity arises from the fact that they are inherently heterogenous and because their surface composition is linked to the environmental conditions that they are in equilibrium with. Fundamental physical chemistry studies provide insights into the detailed molecular processes that underlie the macroscopic phenomena observed on indoor and outdoor environmental interfaces. Importantly, there are enormous benefits to society in delineating the fundamental physical chemistry of these environmental interfaces so as to understand and predict how this chemistry impacts human health as well as the Earth’s climate in the case of atmospheric aerosols.
Acknowledgments
This article gives an overview of the different types of environmental interfaces that have been investigated in the Grassian research group, an area of active inquiry for over two decades. Therefore, the research highlighted here builds on the research conducted by many excellent current and past students and postdocs, a list of all of the individuals involved can be found on the Grassian Research Group website: http://grassiangroup.ucsd.edu.
These distinct areas discussed are funded through different programs. The research that focused on atmospheric aerosols, and sea spray aerosol in particular, is part of research within the Center for Aerosol Impacts on Chemistry of the Environment funded by the National Science Foundation (1305427). The research focused on nanomaterials in the environment is currently funded through the National Science Foundation (1606607). The research focused on indoor surfaces is support by the Alfred P. Sloan Foundation (G‐2017‐9692).
References
- Prather KA, Bertram TH, Grassian VH, Deane GB, Stokes MD, DeMott PJ, Aluwihare LI, Palenik B, Azam F, Seinfeld JH, Moffet RC, Molina MJ, Cappa CD, Geiger FM, Roberts GC, Russel LM, Ault AP, Baltrusaitis J, Collins DB, Corrigan CE, Cuadra-Rodriguez LA, Ebeen CJ, Forestieri SD, Guasco TL, Hersey SP, Kim MJ, Lambert W, Modini RL, Mui W, Pedler BE, Ruppel MJ, Ryder OS, Schoepp N, Sullivan RC, Zhao D. Proc. Natl. Acad. Sci., 2013, 110, 7550-7555.
- Ault AP, Moffet R, Baltrusaitis J, Collins DB, Ruppel M, Caudra-Rodriguez L, Zhao D, Gausco TL, Ebben CJ, Geiger FM, Bertram TH, Prather KA, Grassian VH. Environ. Sci. Technol., 2013, 47, 5603–5612.
- Cochran RE, Laskina O, Trueblood J, Estillore AD, Morris HS, Jayarathne T, Sultana C, Lee C, Stone EA, Lin P, Laskin J, Laskin A, Dowling JA, Qin Z, Cappa CD, Bertram TH, Tivanski AV, Stone EA, Prather KA, Grassian VH. Chem, 2017, 2, 655-667.
- Wang X, Deane GB, Moore KA, Ryder OS, Stokes MD, Beall CM, Collins DB, Santander MV, Burrows SM, Sultana CM, Prather KA. Proc. Natl. Acad. Sci., 2017, 114, 6987-6983.
- Trueblood J, Estillore AE, Lee C, Dowling JA, Prather KA, Grassian VH. J. Phys. Chem. A, 2016, 120, 6444–6450.
- Mudunkotuwa IA, Grassian VH. Environ. Sci.: Nano, 2015, 2, 429-439.
- Mudunkotuwa, IA, Pettibone JM, Grassian, VH. Environ. Sci. Technol., 2012, 46, 7001-7010.
- Gankanda A, Cwiertny DM, Grassian, VH. J. Phys. Chem. C, 2016, 120, 19195–19203.
- Baltrusaitis J, Schuttlefield JD, Zeitler E, Jensen JH, Grassian VH. J. Phys Chem. C, 2007, 111, 14870–14880.
- Weschler CJ, Carslaw, N. Environ. Sci. Technol., 2018, 52, 2419-2428.
- McDonald BC, De Gouw JA, Gilman JB, Jathar SH, Akherati A, Cappa CD, Jimenez JL, Lee-Taylor J, Hayes PL, McKeen SA, Cui YY, Kim SW, Gentner DR, Isaacman-Van Wertz G, Goldstein AH, Harley RA, Frost GJ, Roberts JM, Ryerson TB, Trainer M. Science, 2018, 359, 760–764.
|